Prussian Blue Nanofilm-Sensitized Plasmonic Electrochemical Microscopy For Spatially Resolved Detection Of The Localized Delivery Of Hydrogen Peroxide
- 10 Dec 2024
- Volume 27
- NANOscientific Magazine, FALL 2024
Adaly Garcia, Christina Dhoj, Samuel Groysman, Kinsley Wang, Stellina Ao, Aimee Anguiano, Tony Tran, Dianlu Jiang, Yixian Wang* Department of Chemistry and Biochemistry, California State University, Los Angeles, Los Angeles, CA, USA
This article has been condensed by NanoScientific from the original publication in Elsevier B.V., published online on 26 June 2024, under a Creative Commons Attribution 4.0 International License. This summary aims to provide a shorter and more accessible version of the article. To read the full original article, please visit https://doi.org/10.1016/j.snr.2024.100218.
ABSTRACT
This study introduces a novel Prussian blue (PB) nanofilm-sensitized plasmonic electrochemical microscopy (PEM) technique for real-time, spatially resolved detection of hydrogen peroxide (H2O2). The PB nanofilm, characterized and tested in amperometric mode, effectively visualizes localized H2O2 delivery. Using a micropipette to create and monitor a concentration gradient on the sensor surface, the technique demonstrated precise detection, further validated by numerical simulations. This method holds promise for applications in biomedical research, such as analyzing single-cell exocytosis of neurotransmitters like dopamine, with potential improvements in film uniformity expected to enhance resolution.
Introduction
Traditional electrochemical (EC) techniques typically measure averaged responses from a collection of microstructures, mesoscopic substances, ions, and molecules . While effective for bulk analysis, these approaches o¼en overlook the inherent complexity and heterogeneity of many systems, highlighting the need for EC methodologies capable of spatially resolved detection. Advancements in this area have led to various scanning probe-based imaging methods, such as scanning electrochemical microscopy (SECM) and scanning electrochemical cell microscopy (SECCM), as well as adaptations of optical techniques, including fluorescence, plasmonic , dark field , and Raman scattering microscopy. Scanning probe techniques offer high spatial resolution but o¼en lack temporal resolution, whereas optical methods provide excellent temporal resolution but with reduced spatial information [1-9].
Plasmonic electrochemical microscopy (PEM) emerges as a powerful technique that uses surface plasmon resonance (SPR) on metallic nanofilm electrodes, such as gold or silver, to achieve spatially resolved detection at scales beyond conventional EC approaches. PEM captures local changes due to EC processes on the electrode surface, where plasmonic signals are linked to bulk refractive index changes, dielectric property alterations, redox molecular binding, or EC-induced deposition near the metal film. This microscopy feature enables detailed spatial analysis of electrochemical activities on the electrode [10-22].
In this study, we present a significant advancement in PEM by introducing a "dye-sensitized" approach, termed DS-PEM. This method enhances sensitivity while maintaining precise spatial resolution by coating the PEM sensor with a redox-active dye, which changes color during specific electrochemical reactions, intensifying the optical signals detected by PEM. The technique's model application is the detection of hydrogen peroxide (H2O2), a key biomarker in various physiological processes. Conventional electrochemical assays like amperometry, voltammetry, and impedance spectroscopy are commonly used for H2O2 detection but o¼en lack spatial resolution. Previous spatially resolved detection methods, including microsensor arrays, SECM, and fluorescent imaging, face challenges such as low throughput, laborious protocols, and dependence on fluorescent labeling. [23-29].
The DS-PEM approach addresses these issues by utilizing a Prussian blue (PB) nanofilm as a sensing layer on the PEM chip, building on earlier work that demonstrated the efficacy of Prussian blue nanoparticles (PBNPs) in H2O2 detection via catalytic reduction. In the presence of H2O2, the PB-to-Prussian white (PW) conversion is limited, reducing the refractive index change and the corresponding PEM signal. This inverse correlation allows for a reliable measurement of H2O2 levels. We applied a uniform PB film to the PEM sensor to achieve sensitive, spatially resolved H2O2 detection. The localized H2O2 delivery was simulated using a micropipette, and the resulting micro-scale distributions were mapped using DSPEM, with accuracy validated through numerical simulations. This technique has the potential to become a crucial tool for precise, spatially resolved electrochemical analysis [21, 30].
Experimental Section
Chemicals and Materials
Ferric chloride, hydrochloric acid, hydrogen peroxide, and other chemicals were purchased from Fisher Scientific (Waltham, MA, USA) and used without further purification. Solutions were prepared using double-deionized water from a Milli-Q Ultrapure water system (MilliporeSigma, Burlington, MA, USA). Silicon wells were cut from flexiPERM reusable silicon inserts (Sarstedt, Germany). Electrodes and sensing chips, including goldcoated glass coverslips (18 mm × 18 mm), were obtained from Biosensing Instruments (Tempe, AZ, USA).
Preparation and Characterization of the Prussian Blue Nanofilm
Prussian blue (PB) nanofilms were prepared by depositing Prussian blue nanoparticles (PBNPs) onto cleaned, gold-coated sensing chips. The PBNPs were synthesized by stirring a mixture of potassium ferrocyanide and ferric chloride, and the solution was applied to the sensing chips, followed by overnight baking at 100°C to form a uniform nanofilm.
The surface morphology of the prepared chips was analyzed using atomic force microscopy (AFM). This analysis was conducted in non-contact air mode on a Park NX12 multifunctional microscopy platform (Park Systems, Suwon, South Korea), which is equipped with a detachable AFM head and non-contact cantilevers (PPP-NCHR, 42 N/m, 330 kHz). The instrument was operated using Smart Scan so¼ware, and the AFM images captured were processed with XEI so¼ware, both provided by Park Systems, Suwon, South Korea. Before determining the surface roughness, all images were first-order flattened. The roughness was measured as the root-mean-square deviation of z-heights across the surface within 1 μm × 1 μm sections.
Cyclic voltammetry (CV) was performed using a CHI760E potentiostat (CH Instruments, Austin, TX, USA), showing the expected redox behavior of the PB film. Plasmonic CVs were recorded using the surface plasmon resonance microscopy (SPRm) system from Biosensing Instruments, matching the electrochemical CVs and confirming selectivity for Faradaic processes.
Instrument Setup
PEM experiments were conducted using a surface plasmon resonance microscopy system (SPRm 200 Series, Biosensing Instruments) integrated with a CHI760E potentiostat (CH Instruments, Austin, TX, USA). The electrochemical cell used a threeelectrode setup, with the gold-coated sensing chip as the working electrode. See illustration Scheme 1.
For spatially resolved sensing, H2O2 was locally delivered using a micropipette controlled by a TRIO/MP-245A Micromanipulator System (Sutter Instrument, Novato, CA, USA). The distance between the micropipette and the sensor surface was carefully monitored, and P-polarized light was directed onto the chip to induce plasmonic excitations, with reflected light intensity captured by an SPR detector.
Data Processing
Raw plasmonic images were processed using ImageJ and MATLAB. Data from amperometry tests were background-subtracted and normalized, while cyclic voltammetry data were analyzed by calculating the first-order derivative. Results were based on relative plasmonic signal intensities without converting them to current density values.
Numeric Simulations
Numeric simulations were performed in COMSOL Multiphysics 6.0 to model H2O2 diffusion from the micropipette to the electrode surface. The simulations, which used the “transport of diluted species” module, closely matched the experimental results, validating the accuracy of the technique.
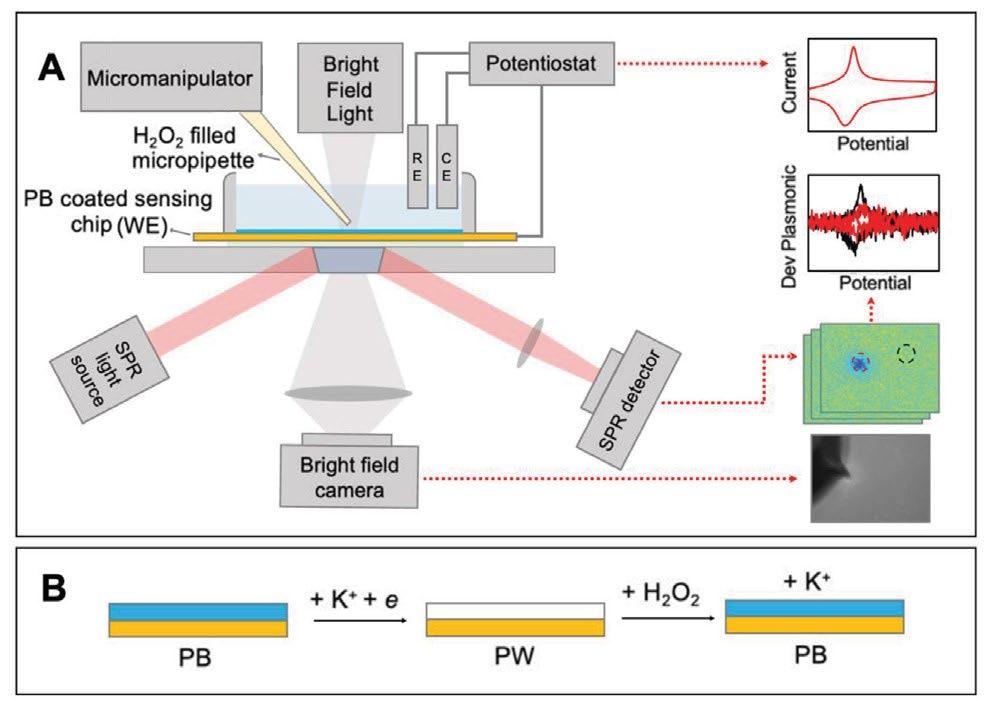
Results and Discussions
Characterization of Prussian Blue Nanofilms
The PB nanofilms were characterized using SEM-EDS, confirming the presence of carbon, iron, and nitrogen, which are indicative of PB's composition (Figs. 1A–C). ATR-FTIR analysis revealed a sharp band at 2075cm-1, corresponding to the cyanide group (Fig. 1G). AFM analysis showed a surface roughness of 9.1 ± 0.9 nm (Figs. 1D–E), with a film thickness of 26 ± 3 nm (Fig. 1F). Electrochemical performance was assessed using cyclic voltammetry (CV), which demonstrated expected redox behavior (Fig. 1H). The plasmonic CV, derived from PEM, matched the electrochemical CV, confirming high selectivity for Faradaic processes (Fig. 1).
elemental mapping of carbon (A), iron (B), and nitrogen (C) confirms the nanofilm's
chemical composition. (D-F) AFM images comparing a bare sensing chip (D) with a
PB nanofilm-coated chip (E), and height profiles (F) at the film boundary. Scale bars:
1 μm for A-E and 0.5 μm for F. Images were first-order flattened using XEI. The inset
in (F) shows height profile extraction points, with an average film height of 26 ± 3 nm
from three measurements. (G) ATR-FTIR spectra of the PB nanofilm (red) and bare chip
(black), highlighting the cyanide peak at 2075 cm¯°. (H, I) Electrochemical analysis with
potentiostat CVs from the whole electrode (~0.9 cm²) (H) and plasmonic CVs averaged from the sensing area (600 μm × 450 μm, 0.27 mm²) (I) for PB-modified (red) vs. bare chips (black) at a 0.05 V/s scan rate in 1 M KNO3.
Sensing Capability of PB Nanofilm-Sensitized PEM
The PB nanofilm's ability to sense H2O2 was tested using amperometry under a constant potential. Plasmonic images showed a decrease in intensity with increasing H2O2 concentration, indicating the catalytic reduction of H2O2 (Figs. 2A–D). Fig. 2F shows the cumulative plasmonic signal from twelve injections, averaged across the sensing area, highlighting its concentration dependence. The plasmonic signal displayed a linear relationship with H2O2 concentration (Fig. 2G), whereas the potentiostat current lacked this correlation at higher concentrations (Fig. 2E). The calibration curve derived from 88 regions of interest (ROIs) exhibited strong linearity (R2 = 0.997), confirming the consistency of the PEM signals for calculating H2O2 concentrations. Comparatively, the signals from bare sensing chips were much weaker and less reliable (Figs. 2H–J).
Sensitized PEM. (A-D) PEM snapshots of the nanofilm at varying
HO concentrations under a −0.1 V bias, taken before each
subsequent injection. Scale bar: 50 μm. (E-J) Real-time data
from the potentiostat (E, H) and PEM (F, I), with calibration
curves correlating PEM signal intensity to H2O2 concentration
(G, J), are shown for both a nanofilm-coated chip (E-G) and a
bare chip (H-J). In F and I, PEM signals are averaged over the
entire imaged area (600 μm × 450 μm, 0.27 mm²), while G and
J present mean ± standard deviation data from 88 regions of
interest (ROIs), each approximately 54 μm × 54 μm. Numbers in
E, F, H, and I indicate H2O2 concentrations in μM, considering
dilution factors.
Spatially Resolved Detection of Locally Delivered H2O2
A micromanipulator system controlled the precise delivery of H2O2 via a micropipette, creating a localized concentration gradient on the sensor surface. Heatmaps generated from PEM images tracked the formation and growth of this gradient as the micropipette approached the sensor (Figs. 3A–E). Concentration profiles extracted from these heatmaps confirmed effective H2O2 delivery and spatial resolution (Figs. 3G, 3H). Real-time concentration variations were observed, with significant increases in the deposition spot (Fig. 3G).
Sensitized PEM. (A-E) Heatmaps showing HO concentrations at the sensing surface
during micropipette approaches under a −0.1 V bias. Scale bar: 50 μm.
Concentrations were determined using the calibration from
Fig. 2. (F) Optical image of the micropipette's position post-approach,
with an inset showing the pipette before use. Scale bar: 20μm.
(G) Distance-dependent concentration traces from specific ROIs marked by dashed
circles in E. (H) Concentration profiles along the x-axis across the delivery site from A-E,
averaged over a 20-pixel span (~20 μm) along the y-axis, at varying micropipette-tosensor distances. (I) Concentration profiles along the x-axis at a fixed micropipetteto-
sensor distance of 0.15 μm, averaged over dierent pixel counts along the y-axis.
(J, K) Comparative plasmonic cyclic voltammograms at the delivery spot (red) versus
an unaected background area (black), shown before (J) and aer taking the time
derivative of the plasmonic signal (K). (L-O) COMSOL-simulated concentration profiles:
2D distribution of HO diusion from the micropipette at distances far from (L) and near (M) the sensor surface (scale bar: 50 μm), and longitudinal concentration profiles across the delivery site at 0.1 s (N) and 1 s (O) aer loading
the micropipette with a pre-set concentration gradient.
The spatial concentration distributions showed clear gradients correlating with the micropipette's distance from the sensor (Figs. 3N–O). Plasmonic cyclic voltammograms (CVs) further validated that the presence of H2O2 resulted in a smaller signal increase at the delivery spot compared to the background (Figs. 3J–K). Numerical simulations using COMSOL Multiphysics supported these experimental results, showing a strong correlation between simulated and observed H2O2 concentrations (Figs. 3L–M).
Conclusions
We successfully developed a novel Prussian blue (PB) nanofilm-sensitized plasmonic electrochemical microscopy (PEM) technique for precise, spatially resolved detection of hydrogen peroxide (H2O2 ). The PB nanofilm was carefully annealed onto the sensing chip, and its ability to detect H2O2 was validated in amperometric mode, showing a clear correlation between the plasmonic signal and H2O2 concentration. By integrating a micromanipulator system, we achieved localized delivery of H2O2, enabling detailed mapping of concentration gradients at the micrometer scale. The accuracy of the PEM technique was further confirmed through numerical simulations, establishing its potential as a powerful tool for exploring localized electrochemical activities. Given PB’s proven effectiveness in catalyzing dopamine, this technique holds significant promise for advancing research in single-cell exocytosis, particularly in studying neurotransmitters like dopamine. Overall, our work extends the applications of PB-sensitized PEM and represents a significant step forward in electrochemical sensing, with promising implications for future bioanalytical research.
REFERENCES AND SUPPLEMENTARY MATERIALS
Supplementary material associated with this article and list of references can be found at https://doi.org/10.1016/j.snr.2024.100218